The economics of fossil decarbonisation in industrial processes for a targeted policy design
Fecha: septiembre 2023
Fossil descarbonisation, Policymakers, Industrial sector
Timo Guerres*
Papeles de Energía, N.º 22 (septiembre 2023)
Current industrial processes, especially those in the basic material sector, rely on fossil fuels and feedstock. Hence, transforming the industrial sector with low-emission processes for a climate-neutral economy implies profound changes. In this paper, I present a conceptual understanding of the economics of fossil decarbonisation by categorising modifications to currently used technologies and differentiating them based on their known and uncertain investment and operational costs. Based on various case studies, I show how different uncertainty dimensions, and the evaluation of uncertainties impact the business case for low-emission process modifications. These uncertainties must be addressed by an industrial policy that guides the transition of the industrial sector. Hence, this work may support policymakers and academia in evaluating and designing targeted policy solutions that guide the industry towards fossil decarbonisation.
1. INTRODUCTION
Most manufactured goods are made from one or several fossil carbon-based basic materials.1 Plastics and other petrochemicals are directly made from crude oil. Coal or natural gas is needed to make carbon steel. We make cement by burning fossil limestone, and lime and other carbon-based minerals play an important role in the ceramics and glass industry. These basic materials have in common that today’s processes are highly standardised, rely on fossil fuels to reach high reaction temperatures, and cause energy-related and process-related carbon emissions. For a climate-neutral economy, these industries must transform completely to eliminate about 60% of the direct industrial emissions caused by basic material production (IEA, 2021) and manufacture final products with a negligible indirect carbon footprint (scope 3) (Hertwich and Wood, 2018).
Nearly all basic materials are commodities, while many intermediary goods made from basic materials are commodity-like. Basic materials are standardised products with clearly defined material characteristics and compositions traded on global markets.2 Hence, for basic material producers, there is little differentiation other than production costs when selling their products. The same holds for a large volume of intermediary goods, such as steel rods or premanufactured concrete structures for the construction industry. Only further down the value chain, product quality, performance, and nontangible characteristics such as design significantly increase the perceived value of a product while diminishing the weight of material and operational costs for the economics of industrial production. As such, the economics of fossil decarbonisation in the industry is primarily linked to the cost competitiveness and profitability of low-emission process modifications compared to current production routes.
My objective is to provide a conceptual understanding of the economics of fossil decarbonisation under consideration of various potential process modification options.
In the following, I introduce the underlying problem description of production costs per unit of product in industrial processes, differentiating between plannable and uncertain production costs (section 2). I then identify four types of low-emission modifications to current processes and highlight how they differ concerning their emission reduction potential and production cost uncertainties. Starting from multiple highly simplified case studies (section 3), I show how cost uncertainty dimensions vary for each type of process modification and how a firm’s investment decision is subject to the evaluation of future uncertain operational cost scenarios. Findings highlight how the attractiveness of investments in low-emission processes can be impacted by uncertain operational costs (section 4). To guide the transformation of the industrial sector towards a climate-neutral economy, industrial policies may have to address operational cost uncertainties in addition to reducing known costs.
2. CONCEPTUAL PROBLEM DESCRIPTION
Any industrial process can be characterised by its production cost per unit of the final product.3 The cost elements that make up the expected total cost per final product can be grouped into costs related to the assets of the physical production process and costs linked to the inputs needed and outputs caused when producing one unit of the final product. This formulation only includes the cost elements expected when investing. It may not correspond to the actual production costs, given the risk of unforeseen outages, equipment malfunctioning, and other cost overruns due to external factors. Outputs can be desired (final product) or undesired (emissions, waste, etc.).

Asset-related costs encompass the investment costs (CAPEX) that need to be recovered over the design life of the process equipment and the operational service and maintenance costs that occur independently from the actual usage of the process but must be allocated to each unit of the final product as well (OPEXinvestment). Theoretically, both cost elements are known for the entire lifetime of the process equipment. They can be allocated to each unit of the expected final product manufactured with the process equipment. In accounting terms, the asset-related costs can be considered fixed costs that are depreciated based on the equipment’s expected service life and use (Hill, 1999).4
Investment costs per unit of product are determined based on the lifetime/depreciation period and the weighted cost of capital (WACC). The longer the depreciation period, the lower the cost per unit of product; the lower the WACC, the lower the cost of the product. The years to recover the investment should not exceed the design life of the process equipment.

Most operational costs are linked to the actual production output (OPEXvariable). For each unit of the final product, a certain amount of input materials and energy is needed. In most cases, materials and energy must be bought on external markets. Their future price is uncertain when investing in new process equipment.5 In addition to the costs associated with the process inputs, operators may face additional costs for undesired outputs linked to the production process operation. In the case of CO2 emissions as an undesired output, prices can be subject to market dynamics. For the EU ETS in Europe, future CO2 prices are highly uncertain when investing in new processes. Other process outputs, for example, rejects or certain waste materials, may create profits (negative costs) that depend on uncertain selling prices.6
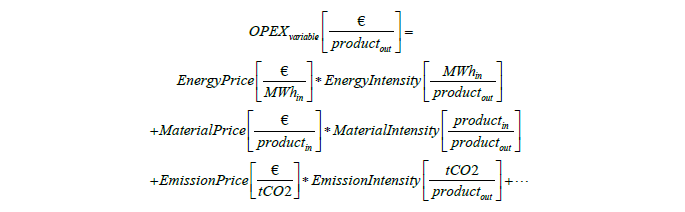
The inputs needed and outputs created per unit of the product depend on the characteristics of the installed process equipment. Hence, energy intensity, material intensity and emission intensity of production are known when investing in process equipment, though emission intensity is a sum-product of both energy and material efficiency.
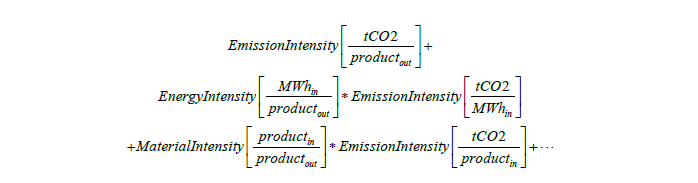
When investing in a new process equipment asset, a firm needs to weigh the known process parameters and asset-related costs against uncertain external market-based prices, among others, for energy, materials, and emission allowances (if subject to a carbon market).
The decision to install novel or modify existing process equipment will depend on the future cost of operating and reinvesting in current equipment. For different types of process modifications, the level of uncertainty changes significantly. In the following, I identify four different types of modification, each with a different degree of future operational cost uncertainty.
Modifications can be grouped into one or several of these four categories of operational cost uncertainty:
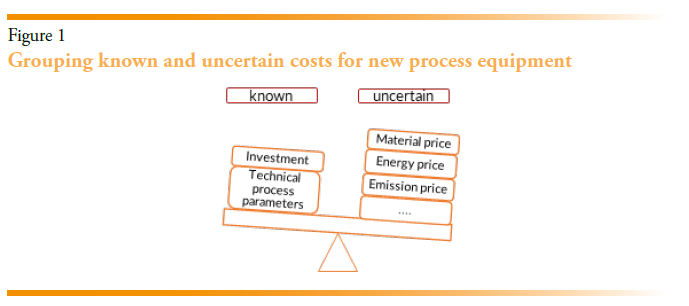
2.1. Efficiency improvements
Process equipment with reduced energy, material, or emission intensity for each unit of the product has a higher efficiency than the current process setup. More is produced with less. Among others, more efficient processes can be achieved with better process control, more efficient use of waste heat or better insulation. Such measures help bring a current process design closer to its thermal optimum and can often be implemented by maintaining the current process and improving it. However, optimising fossil-based processes will not lead to fully eliminating fossil fuels or decarbonising industrial emissions.
From an economic perspective, the main barrier to implementing efficiency improvements is the required investments which must be justifiable by future cost savings for energy, materials, or emissions. Hence, investments in process efficiency must always be justified by future material, energy, and emission cost expectations. The following main uncertainty dimensions for investments in efficiency improvements exist in case energy or material efficiency is being improved:

2.2. Energy carrier change
Only a few industrial processes may operate with another energy source without major modifications.7 Most thermal process equipment is designed for one specific type of fuel or electric energy to produce heat within a certain temperature range and flue gas composition in case of combustion. Hence, changing the energy carrier in an industrial process mostly implies major investments. In some cases, another energy carrier improves energy efficiency, such as using industrial electric heat pumps instead of natural gas boilers. In others, the energy intensity may increase, for example, when using biomass instead of fuel oil.
Given that changing the energy carrier does not impact the process efficiency, the economics of recovering the investments in new process technology or process modifications depend on the following uncertainties. Firstly, the future price of the newly used energy source is not known. However, a firm modifying its processes will still compete with operators of current processes and their operational costs using the conventional energy source with a different emission intensity than the alternative energy source. As such, the economics of the modified process also depends on the uncertain future cost of the conventional energy source and its known emission intensity. The following main uncertainty dimensions for the economics of investments in an energy carrier change exist:
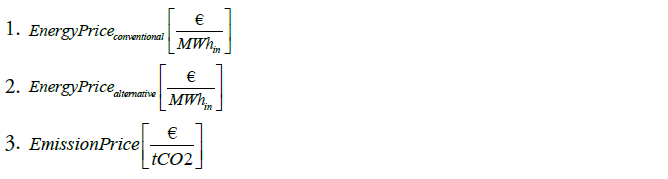
2.3. Processing emissions (or other secondary process outputs)
Capturing CO2 emissions is the most relevant process modification in this category, given the need to reduce the industrial carbon footprint. The economics of carbon capture, though, are very similar to those of any other type of process modification that reduces other greenhouse gases such as methane or nitrogen oxides, as well as investments for the treatment of other secondary process outputs and externalities such as wastewater. Capturing CO2 is an additional non-spontaneous separation process that always requires additional energy, thereby reducing the overall energy efficiency of the process and increasing operational costs.8 A carbon capture process is additional to the operation of the current process. Hence, the energy use for the current process may be the same, while the capturing process relies on another energy source.
The profitability of operating a carbon capture process depends fundamentally on emission savings being financially compensated to recover the additional investment and higher energy costs. Compensation can be indirect by competitors using current processes without carbon capture paying a higher CO2 price. The following main uncertainty dimensions for the economics of investments in carbon capture technologies exist:
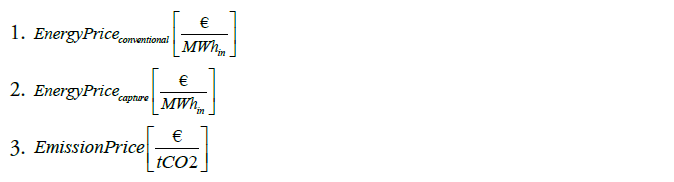
2.4. Input material change
Industrial emissions may result from processing carbon-based input materials, such as in the steel, cement, or petrochemical industry. These emissions cannot be reduced by changing the energy carrier but require the replacement of input materials. Replacing one input material with another changes the characteristics of the final product and the process characteristics. Hence, the energy consumption and emission intensity of the process modification will differ from the current process’s intensities.
Compared to the operation of a current process, at least the following main uncertainty dimensions for the economics of a material change exist:
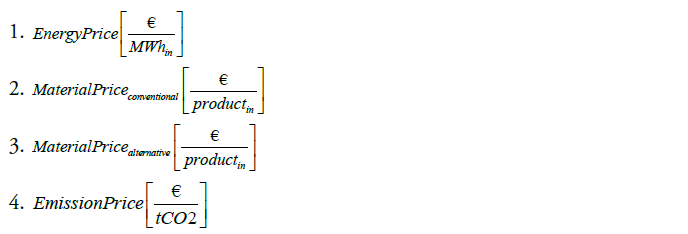
3. CASE STUDIES: THE IMPACT OF UNCERTAINTIES
The expected profitability and the attractiveness of modifying the current process design will always depend on how a firm evaluates the uncertainty dimensions of an investment. However, the impact of each uncertainty dimension on a firm’s ability to recover investment costs while remaining competitive with current processes differs.
In the following, I explore how different expectations on the various uncertainty dimensions may influence a firm’s decision to invest in new or modified process equipment instead of keeping conventional technologies for the four types of process modifications.
3.1. Efficiency improvements: the role of energy and emission prices
The following case represents an investment in any efficiency improvement measure with the new process efficiency exceeding 100% of the current process efficiency consuming the same energy source as the current process. The following case represents an investment in any efficiency improvement measure consuming the same energy source as the current process (insulation, process control, etc.). Given a sample process with a benchmark energy efficiency of 100%, an energy cost of 20 €/MWh and an energy-related emission intensity that corresponds to the use of natural gas, I evaluate three marginal efficiency improvement scenarios (1%, 2% and 3%) assuming a weighted cost of capital (WACC) of 8% per year.
As shown in figure 2, there is an inverse logarithmic correlation between the depreciation period and expected energy prices. Longer depreciation periods for investment increase the total financing costs but lower the investment cost per unit of product produced. Hence, marginally higher efficiency improvements reduce the expected payback period for the investment logarithmically for a constant expected energy price. Higher energy prices reduce the payback period logarithmically.
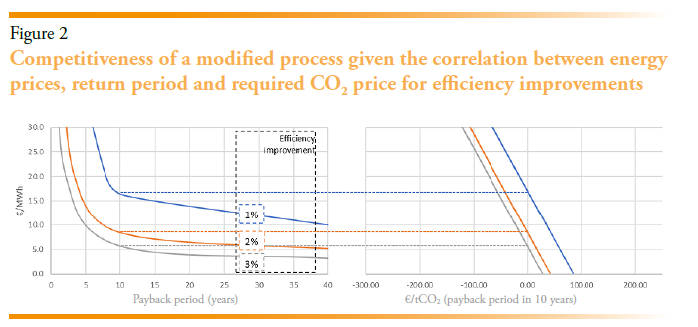
If the efficiency improvement is not economically feasible, a CO2 price can help to ensure investment return. Since the energy carrier remains the same, the required CO2 price corresponds to the difference between the required energy price to make the investment competitive and the actual energy price. figure 2 shows the correlation between energy price and required CO2 price is linear.
3.2. Energy carrier change: emission intensity vs. pricing
The following case represents switching from an emission-intensive energy source, such as natural gas, to a less emission-intensive alternative. Given a sample process with an energy efficiency of 100%, an energy cost of 20 €/MWh for both energy carriers, an energy-related emission intensity that corresponds to the use of natural gas for the conventional energy carrier and a reinvestment cost of 100 €/kW, I evaluate six marginal reinvestment costs scenarios (-1% to - 6.0%) and six marginal energy cost scenarios (+1% to +6%) assuming a weighted cost of capital (WACC) of 8% per year and a depreciation period of 10 years.
Results show a reverse exponential correlation between the emission intensity reduction using an alternative energy carrier and the required CO2 price to make the fuel switch economically feasible (figure 3).
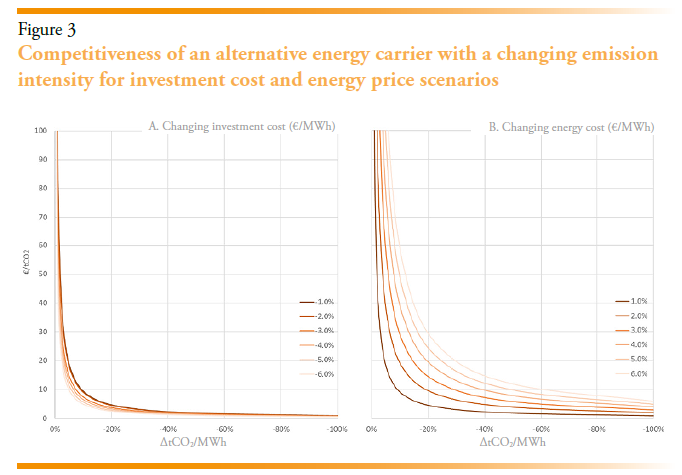
The less emission-intensive the alternative energy carrier, the lower the CO2 price premium needed to make it competitive. However, the non-linearity of the reverse correlation means that additional emission reductions of low-emission processes may only marginally reduce the CO2 price needed to return the investment. Hence the impact on the relative carbon pricing risk is only marginal but increases exponentially with more emission-intensive alternatives.
Reducing the initial investment cost for process modifications lowers the required CO2 price, while higher expected energy costs for the alternative energy carrier results in higher CO2 prices needed to return the investment. Both changes impact the balance between plannable investment cost and uncertain energy cost components per unit of the produced product. For the sample case, the weight of energy cost in the product cost is clearly greater than the capital cost since a marginal increase in the alternative energy cost impacts the required energy price more than a marginal reduction in investment costs (figure 3). However, this result is highly sensitive to the role of investment and energy costs for the final production cost. By doubling the initial investment costs for the sample case (200 €/kW) while maintaining an energy price of 20 €/MWh, the impact of a marginal 1% change of energy or investment costs on the required CO2 price is the same (table 1).
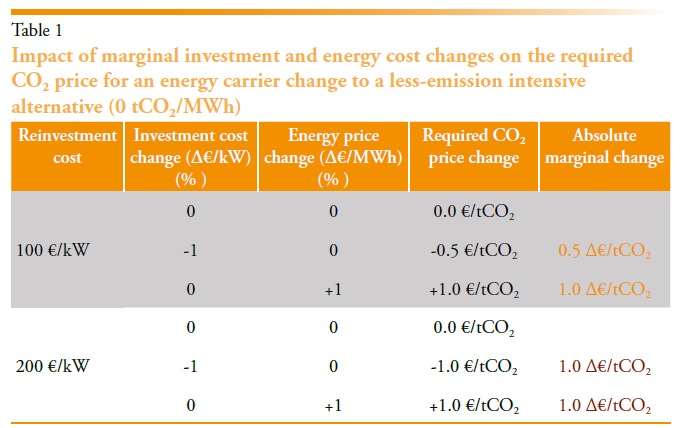
The weight between investment and energy costs is specific to each process and its framework conditions, such as energy costs and availability. Other factors, such as a higher efficiency of heat pumps, can significantly increase the importance of known investment costs compared to uncertain energy costs.
3.3. Processing emissions: why capture requires a CO2 price and high capture efficiencies
The following process is representative of carbon capture installations. I consider a sample process with a required reinvestment cost of 130 €/t of annual production capacity with an energy consumption of 1 MWh per final product and an energy efficiency of 100%, an energy cost of 20 €/MWh and an energy-related emission intensity that corresponds to the use of natural gas. The required investment in the capture process is valued at 35% of the reinvestment, while operating the capture process increases energy demand by 20%. I analyse how variations in the capture efficiency impact the required CO2 price scenarios given the depreciation of the investment within 10 years (WACC: 8%).
As shown in figure 4, the proposed capture technology will not be economically feasible with a CO2 price below 50 €/tCO2, assuming a capture efficiency of 100% and an energy price of 20 €/MWh. Hence, approximately 50 €/tCO2 captured must be “earned” to compensate for additional energy expenditures and the higher investment costs to compete with current processes in this scenario. A lower capture efficiency increases the required CO2 price exponentially. In this example, lowering the capture efficiency from 90% to 89% increases the required CO2 price by 0.85 €/tCO2 while lowering capture efficiency from 20% to 19% hikes up the required CO2 price by 577.55 €/tCO2. Like energy efficiency improvements, the investment in capture carbon technologies is only economically feasible if the yield is sufficiently high. Lower investment or energy costs cannot change this exponential correlation, though they may move the curve closer to a required CO2 price of 0, thereby reducing carbon pricing exposure.
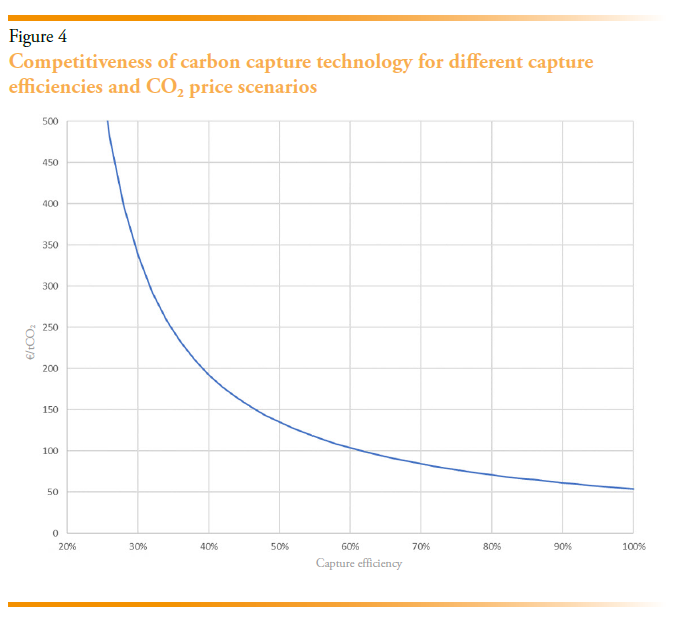
3.4. Input material change: adding another uncertainty dimension
The dynamics between energy carriers and input material change are highly similar. In both cases, the input to the process is changed. What makes input material change different is its implication for process efficiency and energy use. Suppose alternative input materials have diverging material characteristics compared to current process inputs. In that case, the energy efficiency of the process changes and the currently used energy source might not be suitable anymore. Since these dynamics have been described before, I don’t present a separate sample case. Regardless, I conclude that recovering the investment costs for marginal changes of input materials is prone to the highest degree of operational cost uncertainties to evaluate their competitiveness.
4. DISCUSSION: POLICY IMPLICATIONS
Whether or not an investment decision in low-emission process modifications is favourable depends to a high degree on uncertain future operational costs. In industries with highly standardised current processes, reinvestment in these standardised processes seems the safest option since a firm will remain exposed to the same operational cost uncertainties as its direct competitor. Among the discussed process modifications, investments in efficiency improvements are the most attractive choice for a risk-averse firm since, in addition to the fixed costs, the return on investment only depends on the uncertain price development of conventional energy carriers. However, edging closer to the optimal efficiency of current fossil-based processes will not get us to a climate-neutral economy. Fossil fuel use, energy and process emissions remain. We need industries to switch fuel sources, explore different input materials and capture the remaining fossil carbon emissions.
Low-emission process modifications are subject to a wider array of future operational cost uncertainties. Some may argue that, especially for energy costs, a clear correlation between electricity, natural gas and crude oil prices has been observed historically (Batten, Ciner and Lucey, 2017; Zakeri et al., 2022). Such correlations would mitigate uncertainties but cannot be taken as a given. Electricity prices correlate with natural gas prices if natural gas is used for electricity generation, thereby defining the electricity market price. Higher penetration of renewable energy sources making natural gas for electricity generation obsolete, may decouple these energy prices (Gerres et al., 2019) and make the correlation much more latent. By choice, firms that sign long-term power purchase agreements (PPAs) for renewable electricity already decouple themselves from natural gas price developments.
While long-term energy price differences may lead to convergence due to technological adoption, short- and medium-term price fluctuations for different energy carriers would not correlate. However, even in the short or medium term, price fluctuations may have a devastating effect on business, as in the European energy crisis following the Russian invasion of Ukraine in February 2022 (Albert et al., 2022). Similar observations can be made for biomass as a potential substitute for fossil-carbon-intensive feedstock such as coke. If increasingly used as an energy carrier and feedstock, biomass demand may increasingly exceed availability in Europe, resulting in scarcity-driven market prices (Material Economics, 2021). At the same time, uncertainty about the future emission allowance prices on the EU ETS represents an additional cost uncertainty with varying effects in each industry. The resulting varying degrees of uncertainty for different technology options are summarised in table 2.
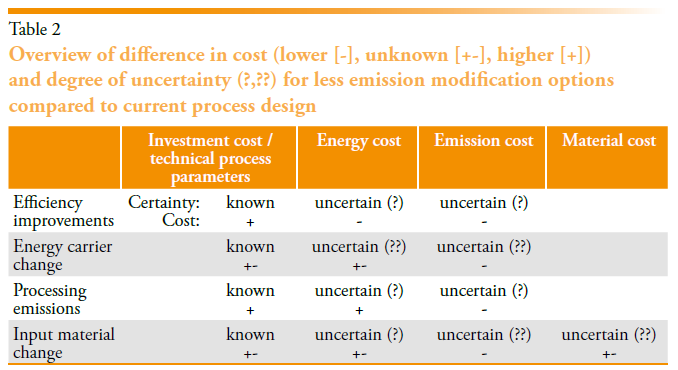
In this context, an industrial policy mainly focusing on investment support may only incentivise process modifications with a high share of fixed costs. Doing so would fail to trigger and guide the transition of industrial processes towards fossil decarbonisation. Instead, policies are needed that address operational cost uncertainties: the OPEX dilemma. Various policy options that could form an industrial policy package have been discussed in the literature (Chiappinelli et al., 2021). Policymakers are tasked to find the right balance between push and pull policies (Corniani, 2008). Industry-specific push policies, such as Carbon Contracts for Differences indexed to energy prices (Gerres and Linares, 2022), should be combined with demand-side pull policies, such as Green Public Procurement (GPP).
5. CONCLUSIONS
Limiting emissions of industrial processes that rely on fossil fuels and feedstock can take different forms. My analysis offers a conceptual understanding of the economics of fossil decarbonisation by categorising modifications to currently used technologies and differentiating them based on their known and uncertain investment and operational costs. Based on various case studies, I show how different uncertainty dimensions, and the evaluation of uncertainties impact the business case for low-emission process modifications. These uncertainties must be addressed by an industrial policy that guides the transition of the industrial sector.
Our work doesn’t offer solutions for such a policy package. It presents the conceptual economic understanding necessary to evaluate and design targeted policy solutions that guide the industry towards fossil decarbonisation. Findings allow for some general observations that may guide policymaking. Policies must acknowledge the different roles of uncertain operational costs depending on the functional principles of process modifications and the weight of known fixed costs compared to uncertain operational costs. Direct investment support might be sufficient to incentivise process modifications with a high share of fixed costs, such as efficiency improvements and industrial heat pumps. If uncertain operational costs outweigh fixed costs, policies should bridge the gap between the operational cost of modified and current processes. Direct support may take the form of Contracts for Differences, indexed against energy prices if energy prices represent the greatest operational cost uncertainty.
REFERENCES
Albert, E., Boutelet, C., Chastand, J.-B., Hivert, A.-F., Stroobants, J.-P. and Madeline, B. (2022). Europe’s energy crisis risks forcing factories across the continent to relocate or close down. Le Monde, Oct. 12, 2022. Accessed: Nov. 02, 2022. [Online]. https://www.lemonde.fr/en/europe/article/2022/10/12/europe-s-energy-crisis-risks-forcing-factories-across-the-continent-to-relocate-shut-down_6000015_143.html
Batten, J. A., Ciner, C. and Lucey, B. M. (2017). The dynamic linkages between crude oil and natural gas markets. Energy Economics, vol. 62, pp. 155–170. DOI: 10.1016/j.eneco.2016.10.019
Chiappinelli, O. et al. (2021). A green COVID-19 recovery of the EU basic materials sector: Identifying potentials, barriers and policy solutions. Climate Policy, May 2021, pp. 1–19. DOI: 10.1080/14693062.2021.1922340
Corniani, M. (2008). Push and Pull Policy in Market-Driven Management. Symphonya. Emerging Issues in Management, no. 1, pp. 45–64. DOI: 10.4468/2008.1.05corniani
Gerres, T. et al. (2019). Rethinking the electricity market design: Remuneration mechanisms to reach high RES shares. Results from a Spanish case study. Energy Policy, Vol. 129, June. p. 11. DOI: 10.1016/j.enpol.2019.03.034
Gerres, T. and Linares, P. (2022). Carbon Contracts for Differences (CCfDs) in a European context. The Greens/EFA, Climate Strategies, 2022. [Online]. http://gruenlink.de/2l3a
Hertwich, E. G. and Wood, R. (2018). The growing importance of scope 3 greenhouse gas emissions from industry. Environ. Res. Lett., vol. 13, no. 10, p. 104013, Oct. 2018. DOI: 10.1088/1748-9326/aae19a
Hill, P. (1999). Capital Stocks, Capital Services and Depreciation. OECD.
IEA. (2021). Industry direct CO2 emissions in the Net Zero and Announced Pledges scenarios, 2000-2030. Oct. 21, 2021. https://www.iea.org/topics/industry (accessed Oct. 03, 2022).
Material Economics. (2021). EU biomass use in a net-zero economy. [Online]. https://materialeconomics.com/latest-updates/eu-biomass-use
World Bank. (2021). World Bank Commodities Price Data (The Pink Sheet). Accessed: Feb. 22, 2021. [Online]. Available: http://pubdocs.worldbank.org/en/804991612306143358/CMO-Pink-Sheet-February-2021.pdf
Zakeri, B. et al. (2022). Energy Transitions in Europe – Role of Natural Gas in Electricity Prices. SSRN Journal, 2022. DOI: 10.2139/ssrn.4170906
NOTES
* Institute for Research in Technology (IIT), ICAI, Comillas Pontifical University, Madrid.
1 Paper pulp, wood products and the food industry are some of the exceptions.
2 See list of commodities according to the World Bank’s “The Pink Sheet” (World Bank, 2021).
3 All mathematical formulations can be representative for variables and parameters in matrix form to reflect the complexity of industrial processes.
4 Fixed costs are based on the premise that assets are operated as initially planned. In the case of unforeseen outages or underused equipment lifetimes might become longer and depreciation lower (or if production levels are lower than expected, assuming the same lifetime, depreciation per unit would become higher).
5 Even if material and energy are produced with internal resources energy and material price risks remain during the design life of the equipment since the production cost of competitors, hence their production and sales price for a product, is subject to energy and material market price fluctuations.
6 This paper focuses on the potential cost of emitting CO2. However, the formulation can be extended by costs for other externalities, such as the correct disposal of solid waste and wastewater.
7 Cement kilns are one of the few exceptions in the heavy industry and can be operated with a wide range of different combustion fuels.
8 Depending on the capturing technology the material intensity of a process may also increase if the capturing process itself requires input materials, such as MEA solvents or calcium oxide sorbents.